Cardioscopic Evaluation and Qualitative Estimation of Mitral Apparatus in A Quasi-Dynamic State in An Ex-Vivo Swine Heart Model
Received Date:March 23, 2020 Accepted Date:April 08, 2020 Published Date: April 11, 2020
doi: 10.17303/jber.2020.4.103
Citation:Tay Qi Ying (2020) Cardioscopic Evaluation and Qualitative Estimation of Mitral Apparatus in A Quasi- Dynamic State in An Ex-Vivo Swine Heart Model. J Biomed Eng 4: 1-9.
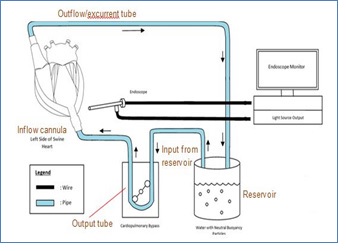
Abstract
Mitral valve disease dominates a significant portion of diseases treated by the cardiothoracic discipline. Prosthetic-related complications such as thromboembolism are postulated to correlate with differences in flow patterns among various prostheses. Methods to observe flow patterns are limited to computational dynamics, cardiac MRI and echocardiography. We describe a novel approach of qualitative estimation of the mitral valve and flow pattern via the use of an endoscope in an ex-vivo swine model. Cardioscopy is utilized along with neutral buoyancy particles in a quasi- dynamic state created by the roller pumps of a cardiopulmonary bypass machine. Direct visualization of the mitral apparatus and flow pattern was achieved via insertion of the endoscope from the ventral surface of the heart and the video recorded was subsequently analyzed with particle tracking software to increase the fidelity of our experiment. Three explanted hearts were used for native mitral valve, Medtronic Mosaic bioprosthetic valve, and Medtronic Open Pivot mechanical valve respectively. Observations include the trajectory of the neutral buoyancy particles, the number of times it encounters the valve as well as the presence of a looped trajectory. Our analysis of the flow patterns concluded that the bioprosthetic valve model was observed to be similar in flow pattern to the native mitral valve while the mechanical valve model deviates from the norm. These results are consistent with the increased risk of post-operative thromboembolism in mechanical valves compared to bioprosthetic valves. This novel approach may find potential in the development and design of new prosthetic mitral valves in the future to emulate the native properties of mitral valve.
Keywords: Cardioscopy; Mitral Valve Apparatus; Swine Model; Mitral Valve Surgery.
Introduction
Mitral valve diseases such as mitral valve regurgitation and mitral valve stenosis dominate a significant portion of diseases treated by the cardiothoracic discipline [1]. These diseases often present with severe consequences and are commonly associated with morbidities such as atrial fibrillation and heart failure. The prevalence of these conditions was reported to be between 5-35% in the United States [1]. The pathology of mitral valve regurgitation is centered around the backflow of blood into the left atrium, from the left ventricle. Often, the coaptation and apposition of the mitral valve apparatus are not ideal owing to certain pathologies affecting the mitral apparatus. The regurgitant jet of blood could be functional or pathological in etiology. In contrast, mitral valve leaflets may either stiffen, thicken or fuse together in mitral valve stenosis. Narrowed mitral valve reduces preload during the diastolic phase of cardiac contraction while increasing the pressure in the left atrium. The myriad of major adverse cardiac and cerebrovascular events such as atrial fibrillation, thromboembolism, congestive cardiac failure, and stroke have prompted a search for definitive management of both conditions [1].
Mitral valve replacement as a definitive treatment modality halts disease progression and restores the cardiac function [2]. Current valve replacement options include either mechanical-biological prostheses [2]. Bioprosthetic valves are derived from the either porcine or bovine origin [3]. Mechanical valves are crafted with materials incorporating pyrolytic carbon, titanium, silicone or steel. The 10-year survival at 68.5% with an early mortality rate at 10.4% [4]. The premise that mitral valve replacement is definitive is challenged by long-term prosthesis-related complications. Thromboembolism is the most common post-operative complication, presenting at 1.5-2.0% per patient-year. Other significant complications include prosthetic valve endocarditis and prosthetic valve degeneration [5, 6].
Endoscopes have been utilized widely for varied purposes, where its main purpose in cardiothoracic surgery has pertained to the visualization of cardiac structures [7]. Endoscopic applications in cardiac procedures have diversified since its inception in the 1920s, where intracardiac observation was done using a rigid endoscope in animal models by Allen et al. [7]. We utilized cardioscopy in our study to observe neutral buoyancy particles flowing through the left ventricle while simultaneously performing a qualitative analysis of the mitral apparatus in a quasi- dynamic state.
Neutral buoyancy particles have an average density equal to the density of the fluid it is immersed in, resulting in an upthrust which balances the gravitational acceleration on the particle. Hence, these particles do not sink nor float and are able to adapt to the established flow pattern, allowing real-time visualization of flow patterns [8]. Neutral buoyancy particles have been applied in fluid dynamics studies with the aid of micro-particle image velocimetry [9-11]. Beyond neutral buoyancy particles, anisotropic particles and red blood cells have been applied as tracer particles [10, 11].
The utilization of both neutral buoyancy particles and cardioscopy has allowed real-time visualization of the flow pattern and mitral apparatus in the left ventricle of an explanted swine heart. Variations in flow patterns may exist between native and prosthetic valves. Disruption of the natural laminar flow pattern in the heart could predispose to various post-operative and long-term complications in mitral valve replacements [5, 6]. The observation of flow pattern and mitral apparatus in a quasi- dynamic state may yet confer a better insight into designing prosthetic valves that simulate the natural laminar flow pattern that we observe with native mitral valves.
Materials and Methods
The ex-vivo experimental set-up is reflected in a schematic fashion in Figure 1. An explanted swine heart was placed on an apparatus with a hole smaller than the width of the mid-section of the heart to expose the ventral surface of the heart. Extra-pericardial fat and pericardium were dissected away using surgical forceps and scissors for proper exposure of the ventral surface and related vessels. The pulmonary veins draining into the left atrium were sutured with 5/0 Premicron PTFE Pledgets to prevent leakage. 2/0 Premicron PTFE Pledgets were sutured tightly around the cannula leading into the apex of the left ventricle. A small incision was made at the apex to connect the inflow cannula to the left apex. The pledgeted sutures were used to anchor the inflow cannula around the cardiac apex. A tube was connected to the inflow cannula and output of the master pump of – Century HLM, LLC Ellisvile, MO 63011 USA cardiopulmonary bypass (CPB) model to establish an inflow of water into the left ventricle from the apex. The end of the tube connecting the apex to the pump is placed into a reservoir of water. Another tube is secured to the ascending aorta and anchored down to act as the excurrent channel for water from the heart, with its other end in the reservoir. Subsequently, the pump was switched to a lower flow to test for any leakage. Any leakage identified would prompt further suturing of the site of leakage after switching off the p.
Endoscopic evaluation of quasi-dynamic system
The roller pump was switched on at a flow rate of approximately 2L/min. A small incision for the endoscope was made in the ventral surface of the left ventricle, approximated to the interventricular septum. An endoscopic light source and camera were attached to a Hopkin’s 10320B rod lens and inserted into the incision, as illustrated in Figures 1 and 2. The endoscope was manipulated to visualize the mitral apparatus and left ventricular outflow tract. Neutral buoyancy particles residing in the reservoir were sucked into the input of the pump and intubated into the heart via the inflow cannula. With the flow established, we observed the coaptation of the mitral valve leaflets as well as the neutral buoyancy particles emulating a flow pattern within the left ventricle. The electrical activity of the explanted heart has ceased and thus no electrical stimulation was provided in this experimental set-up. Externally, the heart appears to be semi- pulsatile. The compliant endocardium and myocardium allowed the pulsatile activity of a heart to be stimulated by the roller pumps. A quasi-dynamic end-diastolic state can be subsequently observed.
Flow pattern evaluation
Blue Cospheric polyethylene microspheres or neutral buoyancy particles were used to visualize the flow pattern of water within the left ventricle. The neutral buoyancy particles had a density of 1g/ml and a diameter of 1mm and are prospected to have a velocity equivalent to particle velocity of water at localized regions [12]. Visual tracking of the trajectory of the colored particles was done in specific regions of the heart via cardioscopy. The flow pattern is estimated to be similar to blood in a live human or animal. A DSLR camera was placed on a tripod stand to record videos of the flow pattern on the monitor. The videos were subsequently extracted and analyzed with software, Tracker Version 5.1.3 (Open Source Physics, Douglas Brown). Each particle was identified and tracked by the software.
Mitral valve replacement
Medtronic Mosaic bioprosthetic mitral valve and Medtronic Open Pivot mechanical mitral valve were surgically implanted into 2 other explanted swine hearts for further experimentation. An incision in the left atrium was made to expose the native mitral valve. A retractor was used to maintain exposure to the left atrium and the mitral apparatus was subsequently excised and removed from the left cardiac chamber. The mitral apparatus would then be cut from the walls of the heart and removed. Mural thrombus present in the left atrium and ventricle were flushed and removed with forceps to provide an unobstructed view for the cardioscope. Figure 3 illustrates the parachuting of a Medtronic Mosaic valve into the left cardiac chamber after the placement of 14 2/0 Premicron PTFE pledgets. The prosthetic valve was then sutured securely with 14 2/0 Premicron PTFE pledgets evenly around the circumference to prevent any instability and paravalvular leak. After the implanted valve was in-situ, 5/0 Premicron PTFE Pledgets was used to suture the incisional access at the left atrium.
Results
Three anatomically similar, explanted swine hearts were used in this study. Each had either a native mitral valve, Medtronic Mosaic bioprosthetic valve or a Medtronic Open Pivot mechanical valve.
The utilization of neutral buoyancy particles allowed successful visualization of the flow pattern in the left ventricle via cardioscopy. A qualitative analysis was also successfully performed using a particle tracking software, Tracker Version 5.1.3. Figure 4A illustrates video snapshots taken at the mitral apparatus. The blue neutral buoyancy particles were visualized clearly as they reflect off the respective valves. Figure 4B corresponds to the analyzed particle trajectory of the respective valves via Tracker Version 5.1.3.
Native mitral valve
The majority of the neutral buoyancy particles seen with the native valve were observed to flow from the apex to encounter the native mitral valve before flowing laterally. This is observed in Figures 4A1 and 4B1. At the lateral wall of the ventricle, the particles were observed to loop and flow towards the left ventricular outflow tract. Particle 1.3 was observed to be an exception, flowing directly towards the left ventricular outflow tract after reflecting off the native mitral valve. Figure 5A illustrates the flow pattern observed in the native mitral valve. The flow pattern was observed to be relatively smooth and shows little deviation from its intended trajectory. The coaptation and apposition of the native mitral valve were observed to be less than ideal. This is attributed to the quasi-dynamic state of the heart and therefore some turbulent flow can be observed, as with Particle 1.3. However, the simulation of the laminar flow in the heart during the end-diastolic state is adequate to illustrate a proper laminar flow pattern in the left ventricle.
Medtronic Open Pivot mechanical valve
Figure 4A2 and 4B2 illustrates the flow pattern observed with the Medtronic Open Pivot mechanical valve. The initial trajectory of the particles was similar to that of the native valve. However, the point at which the particles encounter the mechanical valve was observed to be more lateral. Subsequently, the particles are reflected off the mechanical valve and flowed directly into the left ventricular outflow tract. A looped trajectory similar to the native mitral valve was not observed with the exception of particle 2.4. Apart from particles 2.4 and 2.5, the other particles were observed to follow the general trajectory as described above. Generally, all the particles were observed to randomly encounter the mechanical valve multiple times before flowing towards the outflow tract. Particle 2.4 was observed to have a similar looped trajectory to that of particles 1.1 to 1.5. Particle 2.5 was observed to flow towards the ventral aspect of the left ventricle before reflecting on the outflow tract. Figure 5B illustrates the general flow pattern observed with mechanical valves. The flow pattern in hearts implanted with mechanical valves can be postulated to be random and disruptive, widely varied compared to the natural smooth laminar flow pattern observed with the native valve.
Medtronic Mosaic bioprosthetic valve
Figures 4A3 and 4B3 illustrate the flow pattern observed with the Medtronic Mosaic bioprosthetic valve. The initial trajectory of the particles does not resemble that of either the native or mechanical valves. The course observed was more lateral initially and in the proximity of the bioprosthetic valve the particles converge towards a common point similar to the native valve. The angle of approach is consistent but more obtuse in comparison to the flow pattern in the native mitral valve. As illustrated in Figure 5C, the flow was observed to be laminar and smooth as it encounters the bioprosthetic valve from a lateral approach. The majority of the particles encounter the valve once while few encountered twice. Similar to the mechanical valve model, the flow pattern was not observed to be looped. The subsequent trajectory after encountering the bioprosthetic valve leads directly to the outflow tract. Particles 3.3 and 3.5 were observed to encounter the septal aspect of the outflow tract before leading towards the aortic valve. Generally, the flow pattern observed with the bioprosthetic valve is smooth, with no major deviations. The smooth laminar flow is similar with respect to the native mitral valve, no random and disruptive flow pattern was observed.
The analysis of pattern flow between the 3 different valves has shown significant differences. The flow pattern in the mechanical valve is markedly disruptive and random in comparison to the bioprosthetic and native mitral valve. The flow pattern in the bioprosthetic valve is generally laminar, with minor deviations towards the septal aspect of the outflow tract. Neither bioprosthetic nor mechanical valves had consistent looped flow observed in the native mitral valve model. The particles in the mechanical valve model had multiple encounters with the mechanical valve while the bioprosthetic and native mitral valve had a similar number of encounters. Disregarding the lateral approach and the lack of looped trajectory of the particles in the bioprosthetic valve model, the laminar flow pattern is regarded to be similar to that of the native mitral valve model.
Discussion
Visualization of Left Heart and Flow Pattern by Cardioscopy
Our study has demonstrated the viability of neutral buoyancy particles and cardioscopy in the qualitative estimation of the mitral apparatus and flow pattern. Cardioscopy has enabled 3- dimensional visualization and mapping of flow patterns in the heart. This method confers better resolution and accurate estimation of the flow pattern compared to existing methods such as echocardiography, computational fluid dynamics and cardiac MRI [12-14]. An initial trial of 2- dimensional echocardiography in the study was used to compare with our experimental method. Figure 6 shows an image of 2D echocardiography with less ideal resolution and mapping of flow patterns compared to our proposed method via cardioscopy. Other existing methods utilized echocardiographic particle image velocimetry (PIV) [15]. This method visualizes the direction of flow but is limited to a 2-dimensional space compared to cardioscopy.
Neutral Buoyancy Particles in Studying Flow Pattern
The usage of neutral buoyancy particles in the study of flow in an explanted heart is novel. Flow pattern studies have conventionally relied on computational fluid dynamics, cardiac MRI or echocardiography to analyze the general flow of fluid through the heart. Deviations from trajectories are difficult to detect with current methods [3, 6, 12]. The utilization of neutral buoyancy particles enables individualized particle tracking to analyze how each individual particle flows to estimate the flow pattern. For a detailed analysis of the flow pattern, more neutral buoyancy particles can be intubated to improve the fidelity of the study.
Usage of tracer particles to study blood flow has already been established in the cardiac field [10, 16, 17]. However, these tracer alternatives are not neutrally buoyant and may affect the estimation of the flow pattern accurately. Echo-PIV makes use of micro-bubbles as tracer particle appears to be capable of evaluating the major flow features in the ventricles. However, the bounded spatial resolution of ultrasound imaging limits the small-scale features of ventricular flow and bubbles are not neutrally buoyant and thus may not result in an accurate representation of flow patterns [17]. Special measures also have to be taken to distinguish the intubated bubbles [18]. As such, using neutral buoyancy particles overusing of echo-PIV in our explanted heart model can provide more conclusive and accurate results via cardioscopy.
Potential Effect of Disruptive Flow Pattern on Post-Operative Thromboembolism
Prosthetic valves are thrombogenic in nature. Thromboembolism is one of the most common post-operative complications resulting from prosthetic valves [19]. Mechanical valves, in particular, are more thrombogenic compared to bioprosthetic valves. Bioprosthetic valves exhibit hemodynamic flow similar to native valves and thus such results are certainly expected [3].
Locally turbulent flow causes neointimal injury or dysfunction and the disruptive blood flow in case of atrial fibrillation, atrial dilation or low left ventricular output predisposes to coagulation [20]. In addition, compared to the healthy endothelium constitution in native mitral valves, artificial membranes in prosthetic valves promotes clotting to a greater extent [21].
In our study, the flow pattern observed in the mechanical valve model was not as smooth as examined in the native and bioprosthetic valve. The random and disruptive flow may lead to areas of stasis of blood, predisposing to coagulability. Bioprosthetic valves are also predicted to inherit a lower risk of thromboembolism. This is accrued to the similarities in material and surface endothelium compared to the native mitral valve as well as the smoother laminar flow pattern compared to the mechanical valve model. Our observations were consistent with the theoretical postulations of thromboembolic events in prosthetic valves.
Conclusion
In conclusion, we have developed a novel method of qualitative estimation of the mitral apparatus and flow pattern in the left ventricle. Significant differences in flow patterns and behavior of the mitral apparatus were noted and accounted for in our study. Limitations of our research include the difference in viscosity of water and blood as well as the rigid nature of endoscopes. Procuring neutral buoyancy particles and clear fluids similar to the viscosity of blood could potentially improve the quality of our study. The use of fiber optic or flexible cameras could also enable a variety of maneuvers to completely visualize all intracardiac aspects. Other limitations include the quasi-dynamic state that the heart is in. Natural cardiac contractions produced via the electrical activity of the cardiac nodes would increase the accuracy of our study—changing from a quasi- dynamic state to a completely dynamic state. The use of more prostheses to generate a database on the efficacy of the prosthetic different valves could also be a potential area of research. For further discussion, the development of clear and compliant cardiac models could potentially allow estimation of flow patterns with the naked eye. Nevertheless, our study demonstrates the differences in flow patterns with various prostheses inside the heart. Our methods are relatively easy to reproduce owing to minimal materials used and are therefore a viable method to test and design prosthetic mitral valves.
Acknowledgements
The authors wish to acknowledge valuable support from Dr Dr Chiam Sher-Yi (NUS-HS).
Declaration of conflicting interests
The Authors declare that there is no conflict of interest.
- Freed LA, et al. (1999) Prevalence and clinical outcome of mitral-valve prolapse. N Engl J Med 341: 1-7.
- Rizzoli G, et al. (2010) Valve surgery in octogenarians: does it prolong life? Eur J Cardiothorac Surg 37: 1047-1055.
- Sun JC, et al. (2009) Antithrombotic management of patients with prosthetic heart valves: current evidence and future trends. Lancet 374: 565-576.
- Stahle E, et al. (1997) Long-term relative survival after primary heart valve replacement. Eur J Cardiothorac Surg 11: 81-91.
- Misawa Y (2015) Valve-related complications after mechanical heart valve implantation. Surg Today 45: 1205-1209.
- van der Merwe J and F Casselman (2017) Mitral Valve Replacement-Current and Future Perspectives. Open J Cardiovasc Surg 9: 1179065217719023.
- ALLEN DS & Graham EA (1922) Intracardiac surgery—a new method: a preliminary report. Journal of the American Medical Association 79: 1028-1030.
- Kerho MF & Bragg MB (1994) Neutrally buoyant bubbles used as flow tracers in air. Experiments in Fluids 16: 393-400.
- Homann H, Bec J (2010) Finite-size effects in the dynamics of neutrally buoyant particles in a turbulent flow. Journal of Fluid Mechanics 651: 81-91.
- Lee JY, HS Ji and SJ Lee (2007) Micro-PIV measurements of blood flow in extraembryonic blood vessels of chicken embryos. Physiol Meas 28: 1149- 1162.
- Matisse P & Gorman M (2019) Neutrally buoyant anisotropic particles for flow visualization. The Physics of Fluids 27: 759-760.
- Morris PD, et al. (2016) Computational fluid dynamics modeling in cardiovascular medicine. Heart 102: 18-28.
- Itatani K, et al. (2017) New imaging tools in cardiovascular medicine: computational fluid dynamics and 4D flow MRI. Gen Thorac Cardiovasc Surg 65: 611-621.
- Su B, et al. (2016) Cardiac MRI based numerical modeling of left ventricular fluid dynamics with mitral valve incorporated. J Biomech 49: 1199-1205.
- Faludi R, et al. (2010) Left ventricular flow patterns in healthy subjects and patients with prosthetic mitral valves: an in vivo study using echocardiographic particle image velocimetry. J Thorac Cardiovasc Surg 139: 1501-10.
- Gao H CP, Amzulescu M-S, Stankovic I, D’hooge J, Voigt J-U (2012) How to optimize intracardiac blood flow tracking by echocardiographic particle image velocimetry? Exploring the influence of data acquisition using computer-generated data sets. Eur Heart J Cardiovasc Imaging 13: 490-499.
- Kheradvar A, et al. (2010) Echocardiographic particle image velocimetry: a novel technique for quantification of left ventricular blood vorticity pattern. J Am Soc Echocardiogr 23: 86-94.
- Mele D, et al. (2019) Intracardiac Flow Analysis: Techniques and Potential Clinical Applications. J Am Soc Echocardiogr 32: 319-332.
- Roudaut R, K Serri and S Lafitte (2007) Thrombosis of prosthetic heart valves: diagnosis and therapeutic considerations. Heart 93: 137-42.
- Wolberg AS, et al. (2012) Procoagulant activity in hemostasis and thrombosis: Virchow’s triad revisited. Anesth Analg 114: 275-85.
- Noble S, et al. (2009) Anatomo-pathological analysis after Core Valve Revalving system implantation. EuroIntervention 5: 78-85.
Figures at a glance